Effect of radiations on nucleotide sequence

Fig. 23.28. Relationship of radiation dose with total hits and effective hits (based on target theory).

Fig. 23.29. Relationship of radiation dose with different kinds of chromosomal aberrations involving single breaks and double breaks in cultured human cells. Note that terminal deletions involving single breaks have a linear relationship with the dose, while interstitial deletions and exchanges have a linear relation only with square of the dose.

Fig. 23.30. Relationship of radiation intensity and dose with per cent chromosomal aberrations observed in Tradescantia, showing that highest intensity gives highest frequency of aberrations at higher doses.

Fig. 23.28. Relationship of radiation dose with total hits and effective hits (based on target theory).
The frequency of simple chromosomal aberrations e.g., terminal deletions, is proportional to the dose, since they arise by single hits. More complicated aberrations needing two breaks (interstitial deletions and exchanges), 'increase approximately with the increase of square of the dose (Fig. 23.29). The frequency of two hit aberrations is more if radiation is given continuously than rf given in fractions, since in the latter case healing of the breaks may take place. Intensity of radiation may also influence the frequency of aberrations, which would be higher at higher intensity and will increase with increase of square of the dose (Fig. 23.30). Chloramphenicol has been shown to keep the breaks open longer, showing a role of protein in healing.

Fig. 23.29. Relationship of radiation dose with different kinds of chromosomal aberrations involving single breaks and double breaks in cultured human cells. Note that terminal deletions involving single breaks have a linear relationship with the dose, while interstitial deletions and exchanges have a linear relation only with square of the dose.

Fig. 23.30. Relationship of radiation intensity and dose with per cent chromosomal aberrations observed in Tradescantia, showing that highest intensity gives highest frequency of aberrations at higher doses.
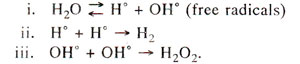

Fig. 23.31. Relationship of ultraviolet radiation dose with mutation rate in Eschcrichia coli, Aspergillus and Neurospora crassa. Note that in Aspergillus, frequency of mutations falls down at higher UV doses.

Fig. 23.32. Hydration of cytosine (C) into hydroxylcytosine (HC), etc., in presence of ultraviolet (UV) rays.

Fig. 23.33. Formation of thymine dimers from the adjacent thymine molecules in a DNA segment due to ultraviolet (UV) rays.

Fig. 23.34. Base pairing between adenine (A) and rare imino form of cytosine (C).

Fig. 23.35.Base pairing between guanine (G) and rare enol form of thymine (T).
In bacteria the possibility of an indirect induction of mutations by UV has been clearly established. UV irradiation of culture media have been found to produce mutagens which induced mutations in unirradiated organisms placed in these media within four hours. The radiation product is apparently unstable and organic peroxides or radicals have been suggested as the cause of mutation. Organic peroxides are mutagenic while H2O2 in saline is not. But since UV also acts on the pyrimidine bases and on the corresponding nucleotides within the bacterium, it is not known, how the mutagenic effect comes about. That most UV induced mutations involve extrachromosomal material is obvious from the fact that their frequency is reduced if post irradiation protein synthesis is blocked or altered. The frequency is similarly increased by addition of nucleic acid bases. The relationship of mutation frequency and UV dose is also not always linear or exponential.
For instance, in Aspergillus, at higher doses the frequency falls down (Fig. 23.31).

Fig. 23.31. Relationship of ultraviolet radiation dose with mutation rate in Eschcrichia coli, Aspergillus and Neurospora crassa. Note that in Aspergillus, frequency of mutations falls down at higher UV doses.
A relationship between UV and DNA could be shown by in vitro studies, where thymine and cytosine get hydrated by addition of a H2O molecule (Fig. 23.32). Similarly two molecules of thymine get connected to give rise to a thymine dimer (Fig. 23.33).

Fig. 23.32. Hydration of cytosine (C) into hydroxylcytosine (HC), etc., in presence of ultraviolet (UV) rays.

Fig. 23.33. Formation of thymine dimers from the adjacent thymine molecules in a DNA segment due to ultraviolet (UV) rays.
Spontaneous mutations are induced under normal conditions of growth. Some of these mutations should originate from mistakes in normal duplication of DNA. Transitions may be produced by tautomeric shift (or ionization) of bases, which leads to mistaken A-C base pairing (Fig. 23.34) and more frequently mistaken G-T base pairing (Fig. 23.35). The transitional changes in both directions can be obtained (Fig. 23.36). Transversions might arise by mistaken pairing between two purines or two pyrimidines. Several such pairs (A-G and C-T) are possible, using single or double bonds. In the latter case they have slightly wrong distances or angles for the two sugar (C)-base (N) bonds. Since DNA backbone is not completely stiff, it can accommodate such distortions, at least occasionally, as a mistake. AT→CG base pair changes may occur due to A-G or T-C base pairing (Fig. 23.37). Similarly GC→TA base pair changes will result from G-A and C-T base pairing (Fig. 23.38).

Fig. 23.34. Base pairing between adenine (A) and rare imino form of cytosine (C).

Fig. 23.35.Base pairing between guanine (G) and rare enol form of thymine (T).
Deletions and additions may also occur spontaneously due to loop formation, when a base may not be copied or may be copied twice.